NeuroNex News
May 2023, Issue #2
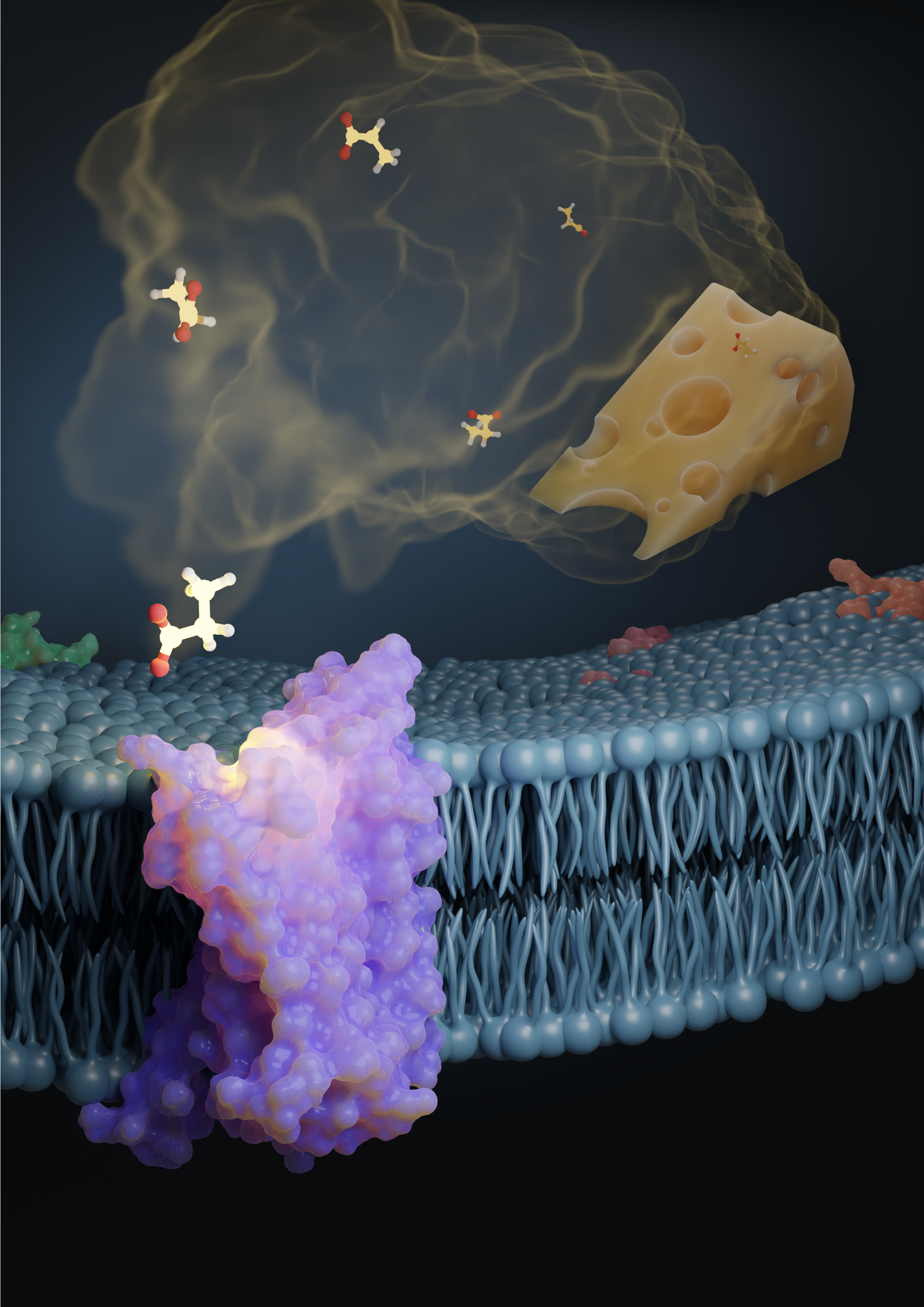
In this illustration, the propionic acid odorant—one of the compounds that give Swiss cheese its distinct smell—activates the odorant receptor protein, OR51E2. Researchers recently discovered how odor receptors are activated in humans and other vertebrate species. Illustration created by Claire de March.
Take a whiff of a hyacinth flower and, in a matter of seconds, the olfactory receptor neurons in your nose pick up the smell; the receptors in those neurons bind to the odor molecules, triggering a nerve impulse that travels along the olfactory nerve to the brain. The olfactory nerve then sends the nerve impulse to the olfactory bulb—a small structure at the base of the brain—which processes the information and sends it to other parts of the brain.
For the first time, researchers have an answer to how these odor receptors are activated in humans and other vertebrate species. As part of this effort, they looked at how odor receptors, which belong to G protein-couple receptors or GPCRs—bind to the odor molecule. They recently published their findings in Nature.
For starters, humans have about 400 receptors, and each receptors is tuned to a specific set of smells. That means that every smell we take in—from freshly cut grass to aged cheese—activates a specific combination of receptors as each odor molecule binds to multiple odor receptor proteins.
In order to study this, however, researchers would first need to study the structure of the odor receptor protein to understand how and why they bind to specific molecules. For that, they rely on the cryo-electron microscopy or cryo-EM technique that allows them to study the 3D structure of proteins and molecules. But there have been two challenges related to this: 1. Most receptor proteins are difficult to produce outside the nose; 2. Researchers have typically needed milligrams of purified protein to accurately determine their structure.
Over the last few years, however, the cryo-EM technique has advanced to a point where researchers need a lot less of the receptor protein—micrograms instead of milligrams—to determine its structure. In addition, as part of this recent effort, out of the 400 or so receptors, researchers identified a single receptor—OR51E2—that’s most efficient in producing proteins, as it turns out not just in the nose but also in the kidney cells. This particular receptor responds to the acids propionate and acetate—produced by our gut bacteria and abundant in our bodies, particularly in our gut cells. “If you think about olfactory receptors, they're obviously expressed in the olfactory sensor neurons and detect odorants, but then some of the receptors are actually expressed in other cells, like gut cells, or kidney cells, or some other places, to sense things in the body,” says Hiroaki Matsunami, author on the study and Professor of Molecular Genetics and Microbiology and of Neurobiology at Duke University’s School of Medicine. He’s also part of the NeuroNex project, Odor2Action: Discovering Principles of Olfactory-Guided Natural Behavior, which is focused on understanding how we detect natural chemical odorants. “That, by itself, is interesting,” says Matsunami. “For us, it indicates that this particular receptor is different because it's not only functioning in the nose, but also functioning in other cells. We thought it has a better chance to be expressed.” Next, researchers looked at why OR51E2 responded to those particular short-chain fatty acids and not, for instance, longer-chain carboxylic acids. They tested this by stimulating OR51E2-expressing cells with a set of carboxylic acids and monitoring the activation of OR51E2. Understanding the structural analysis of the protein was key because it not only helps shed light on how different receptors are tuned but also allows researchers to make mutations to engineer the receptor structure to change its tuning. “We definitely need to experimentally determine more structures, but we are now capable of predicting or modeling the structure of all the receptors,” says Matsunami. Once we have it, it’s possible to computationally screen what kinds of odorants may bind to a particular odorant receptor.” This would help researchers efficiently screen newer chemicals that may be used in perfumes, flavors, or food additives, for instance.
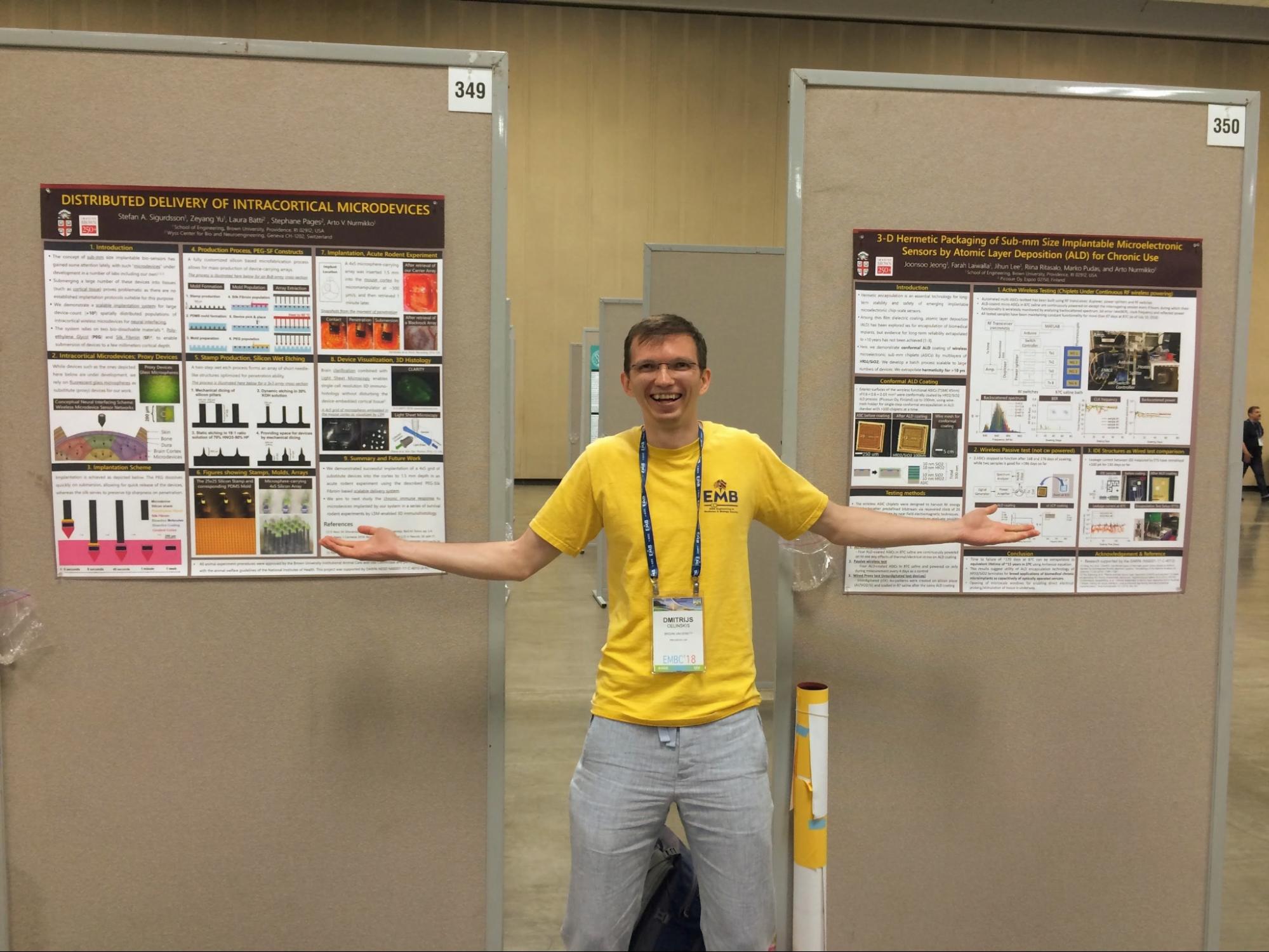
Arms outstretched at the 40th International Conference of the IEEE Engineering in Medicine and Biology Society in Honolulu, Hawaii, Celinskis not only presents his own research on wearable ultrasound sensors used to detect blockages in blood vessels but also that of his labmates who could not attend the conference. Image courtesy of Dmitrijs Celinskis.
For Dmitrijs Celinskis, a researcher with a background in medical physics and biomedical engineering, it’s the complexity of neuroscience combined with the ability to do impactful and meaningful work that appeals to him. “Most things we work on in neuroscience have a real chance for making a future positive impact on human health,” he says. “I appreciate this aspect of work a lot.”
Celinskis recently received his doctoral degree in biomedical engineering, for which he worked with two labs at Brown University—one at the School of Engineering, where he studied the mechanisms of pain and nociception—the physiological way in which the nervous system detects and responds to harmful stimuli. The other was at the Department of Neuroscience, where, as part of the NeuroNex project, Bioluminescence for Optimal Brain Control and Imaging, he explored the use of bioluminescence for novel calcium indicators. These indicators—traditionally composed of fluorescent molecules sensitive to changes in calcium ion concentration within cells—allow neuroscientists to study neuronal activity in real-time, providing key insights into how the nervous system functions.
“One of my ultimate goals is to combine the two areas of my PhD work and apply bioluminescent indicators to gain deeper insights into nociception and pain,” says Celinskis.
As a postdoctoral researcher, he works on the NeuroNex Bioluminesce Project, focusing on molecular mechanisms of sensory information processing. These mechanisms enable detection of events like a pinprick. Celinskis uses tools like two-photon fluorescent microscopy, bioluminescence imaging, and optogenetics to study this molecular machinery's life-supporting functions.
When it comes to pursuing a career path, Celinskis believes in staying open-minded—learning new things and embracing new challenges along the way. He has the same advice for aspiring neuroscientists and engineers: “Seek hands-on opportunities to immerse yourself into what it is that you’re interested in and reflect on how it feels,” he says. He encourages students to explore summer schools, workshops, outreach events, and other opportunities that will provide them with valuable hands-on experience. “Neuroscience in particular is so huge,” he says. “I definitely wouldn’t recommend settling immediately on the first thing that you try.”
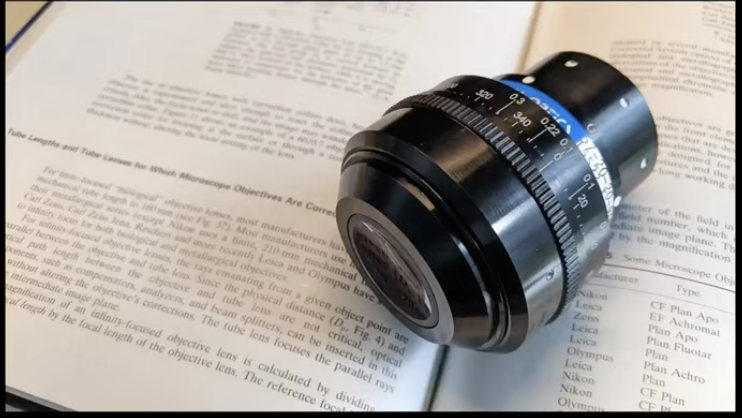
Named after Cousa squash, the Cousa objective is a long working distance air objective for multiphoton imaging in vivo. Image courtesy of Nemonic NeuroNex.
The Nemonic: Next generation multiphoton neuroimaging consortium Project, based at the University of California, Santa Barbara, is developing tools and technology for multiphoton imaging and optogenetics. “This entails engineering novel optics and other instrumentation to expand the field-of-view of multiphoton neuroscience experiments,” says Project PI, Spencer Smith.
Consider, for example, the Diesel2p. Introduced in late 2021, this two-photon microscope can image individual neurons at a cellular-scale resolution across multiple parts of the brain and at an unprecedented scale. The Diesel 2p is open source and has, so far, been sold in at least six different countries around the world including the US, Japan, United Kingdom, and Canada. The team has also developed three-photon and quad-region versions of the Diesel2p. Similarly, the team has released the Cousa objective—the only multiphoton-optimized air objective with 20mm of working distance, a 2-3mm wide field-of-view, and a numerical aperture of 0.5. Unlike water-immersion objectives, it offers a longer distance between the lens and sample. Another open-sourced tool, so far roughly 30 Cousa objectives have been sold.
In recent years, researchers have developed technologies like superresolution multiphoton imaging, ultrafast pulse characterization, and miniaturized multiphoton systems. “The team of investigators have been prolific, creative, and open, leading to tremendous success for this NeuroNex project, and they spawned an array of new exciting projects that are currently underway” says Smith.
For instance, they’re also working to provide centralized and traveling instrumentation for experiments that require complex instrumentation. “Individual PIs may have experiment ideas that are possible but require instrumentation and/or expertise beyond their labs' means,” says Smith. “We can provide that and help execute their experiments in an efficient manner.” The team is also spinning out a company to offer new optical designs that improve fields-of-view, resolution, sensitivity, and time resolution for neuroscientists.